REACTORS TO ROCKETS
The nuclear renaissance has arrived. The case for investment in nuclear for commercial energy on earth is strong. The case for nuclear in space and defense technology is even stronger.
NUCLEAR POWER FOR SPACE AND DEFENSE TECHNOLOGY
The nuclear renaissance has arrived. The case for investment in nuclear for commercial energy on earth is strong. The case for nuclear in space and defense technology is even stronger. There are indications that off earth applications may lead the way. Opportunities for investment abound now.
On earth, a growing world, electrification, and the expansion of artificial intelligence (AI) and data centers will cause dramatic growth in the demand for electricity. A confluence of policy changes, financial support, and technological advances will ensure that nuclear power is a chief supplier of electricity on earth.
Rapid changes in the defense industry, with building mass in autonomous vehicles and their own associated AI and data centers, bring a similar demand for electricity that is continuously reliable and portable. Off earth, the expanding space economy has parallel demand, and additional demand for the benefits of nuclear propulsion systems.
Advances in fission technology are bringing new safe, reliable, and scalable solutions to power generation. Radioisotope power systems for smaller and enduring power sources, previously only made by governments, are poised to be manufactured at scale. Finally, the promise of fusion as a clean and unlimited power source appears closer to reaching fruition.
These technologies and solutions have all drawn significant private capital and government investment via a large number of emerging private companies. Nuclear for space and defense technologies has transformational potential for investors while also providing great benefit to maintaining peace and enabling human expansion in the solar system.
THE NUCLEAR RENAISSANCE
The US government, major financial institutions, and big technology companies have embraced nuclear energy. Recent policy changes, financial commitments, and technological developments confirmed a revitalization of nuclear energy in the United States and globally.
• The recently published report, Pathways to Commercial Liftoff : Advanced Nuclear, by the U.S. Department of Energy (DOE) outlined projected increases in energy demand and the critical role that nuclear energy will play in meeting that demand. The DOE estimates that the United States will need an additional 60 to 95 GW of new nuclear capacity by 2050 to support a decarbonized energy system.
• Our models show that the progression of advanced computing, expanding data centers, and the need to replace retiring coal plants will create a new demand of over 450 GW. Worldwide electricity demand could more than double in the next ten years.
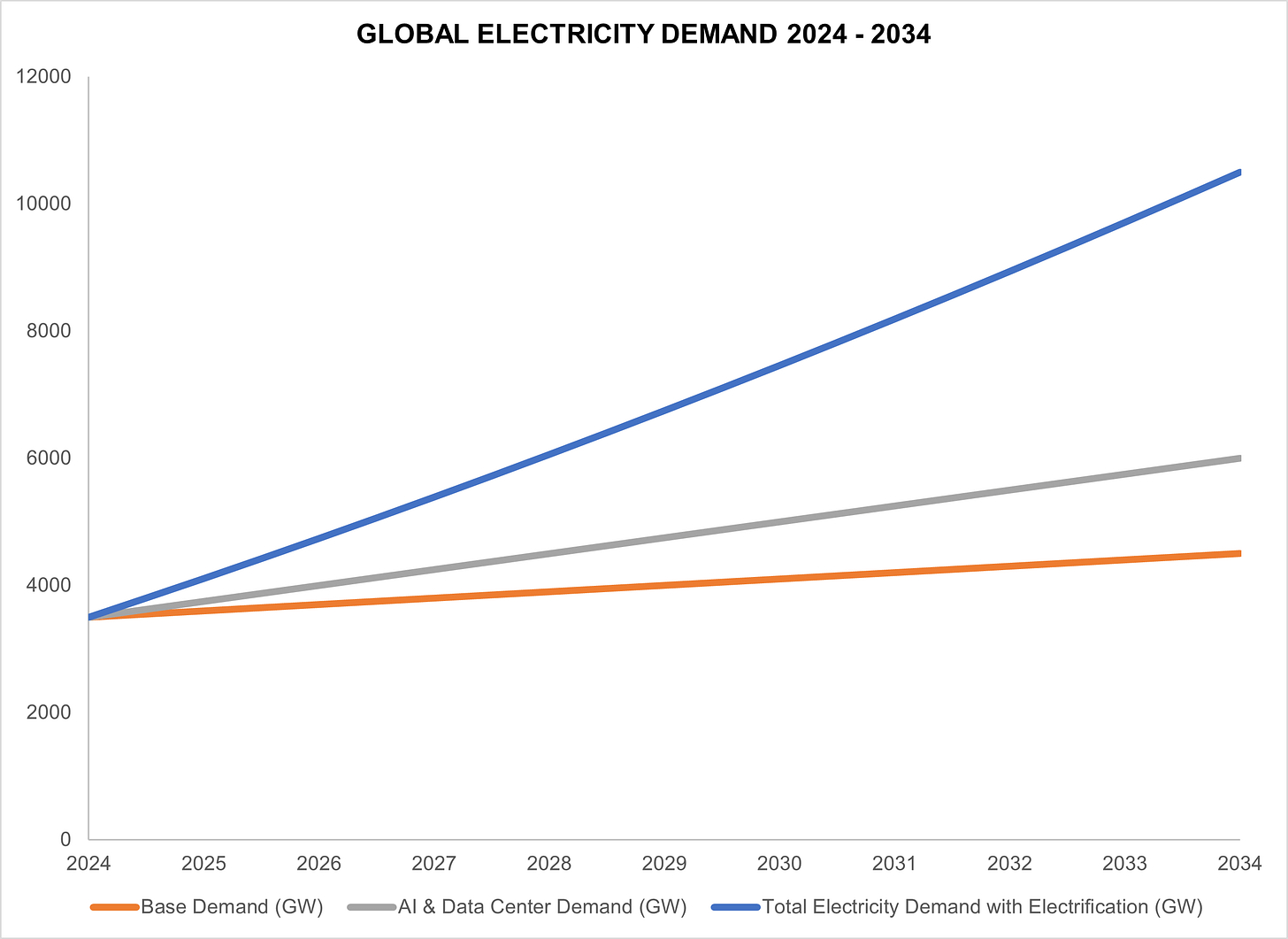
There is concrete policy and financial support from the US government:
• The Inflation Reduction Act (IRA) included substantial tax credits for existing reactors and a 30-50% investment tax credit for new reactor production.
• The FY2024 budget included $2.72 billion to incentivize a domestic nuclear fuel supply chain and $900 million to support new generation SMRs.
• Congress passed the ADVANCE Act to streamline licensing processes.
In addition to providing concrete support, this signaled to the private sector that nuclear is a solid investment.
Fourteen major financial institutions announced during a meeting in New York City that they would begin providing financing for nuclear energy projects. This change in stance was a major departure from previous industry norms where nuclear investments were often perceived as high risk. Now, significant new capital is available for nuclear projects.
• Amazon announced that it will collaborate with Energy Northwest and X-energy to develop four SMRs in Washington. Additionally, it is partnering with Dominion Energy to explore the development of an SMR near an existing nuclear plant in Virginia.
• Google revealed a power purchase agreement with Kairos Power for electricity from multiple SMRs, with plans to bring the first reactor online by 2030.
• Microsoft signed a 20-year agreement with Constellation Energy to restart a dormant reactor at Three Mile Island, expected to resume operations in 2028. The company invested in the nuclear fusion start-up Helion.
The United Kingdom, Canada, and South Korea, are similarly exploring nuclear power as a cornerstone of their energy strategies. China has also been building and approving new reactors. The UAE recently achieved a successful goal of 25% of their electricity provided by nuclear power.
The nuclear industry will meet rising demand with a spectrum of new fission power generation from microreactors, small modular reactors (SMRs), and new versions of traditional large reactors. At the same time, private companies are developing the ability to produce small radioisotope power systems. Finally, over $7 billion in capital has been invested into developing the ability to produce power with fusion.
Beyond serving critical traditional energy needs on earth, nuclear power has a singular role to play in powering the new vehicles and systems in the rapidly changing defense world, and in providing power and propulsion in the extreme environments of the new space economy.
NUCLEAR POWER FOR DEFENSE TECHNOLOGY AND THE NEW SPACE ECONOMY
Nuclear energy has played a transformative role in national defense since its inception from enabling virtually unlimited operational range for submarines to providing reliable power in remote and austere environments. Anticipated future roles include:
• Replacing diesel generators at forward operating bases
• Power supplies for autonomous systems
Likewise nuclear energy was and remains fundamental to the space mission fulfilling the need to power satellites long-term without the need for repowering. Nuclear is anticipated to be a fundamental supply for:
• Future satellites
• Lunar and Martian bases
• Large spacecraft
• Deep space propulsion systems
Nuclear Power for Defense Technology
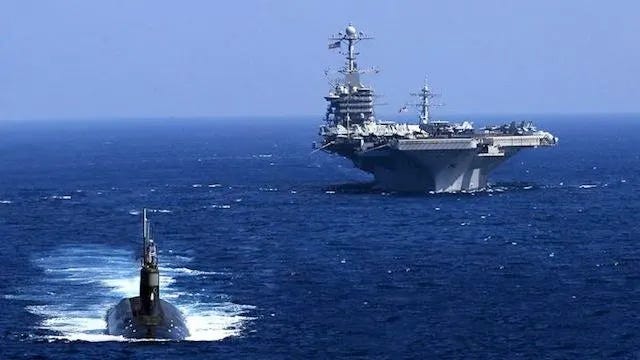
The U.S. Army explored the use of small nuclear reactors for remote power generation during the Cold War through the Army Nuclear Power Program (ANPP). This program deployed several reactors, including the PM-2A reactor in Greenland to power the Camp Century research base and the MH-1A floating reactor to provide power in the Panama Canal Zone. These reactors demonstrated the ability to deliver consistent and reliable power in isolated or forward locations, reducing the need for fuel supply convoys, which are vulnerable to attack.
Forward operating bases (FOBs) rely heavily on diesel generators for power, which presents logistical challenges and operational vulnerabilities. Supplying diesel fuel to FOBs requires extensive logistics and poses signifi cant risks to personnel and equipment in conflict zones. Nuclear microreactors, such as those being developed under the Department of Defense’s Project Pele, are poised to revolutionize power supply at FOBs by providing a compact, reliable, and low-maintenance power source.
These microreactors, designed for rapid deployment and ease of transport, can reduce or eliminate the need for diesel fuel, enhance energy security, and provide uninterrupted power for base operations and communications.
As the battlefield becomes more reliant on unmanned systems, the need for efficient power sources is growing. Nuclear energy, with its high energy density and long operational life, is an ideal candidate for powering and recharging autonomous systems. Mobile nuclear reactors or advanced radioisotope thermoelectric generators (RTGs) could provide a continuous power supply for drone swarms, unmanned ground vehicles (UGVs), unmanned underwater vehicles (UUVs), and autonomous aircraft, enabling prolonged operations without the need for frequent refueling or battery swaps. Nuclear-powered recharging stations could support a network of autonomous vehicles and sensors, providing a resilient and decentralized power infrastructure for military operations.
Beyond powering vehicles, nuclear energy could support strategic mobility by providing power for electromagnetic launch systems, directed energy weapons, and advanced communication systems that require consistent, high-power output. Small modular reactors (SMRs) and advanced reactors could be deployed as power sources for large-scale military bases, offering a secure and resilient energy solution that is less dependent on local grid infrastructure.
Nuclear Power for the New Space Economy
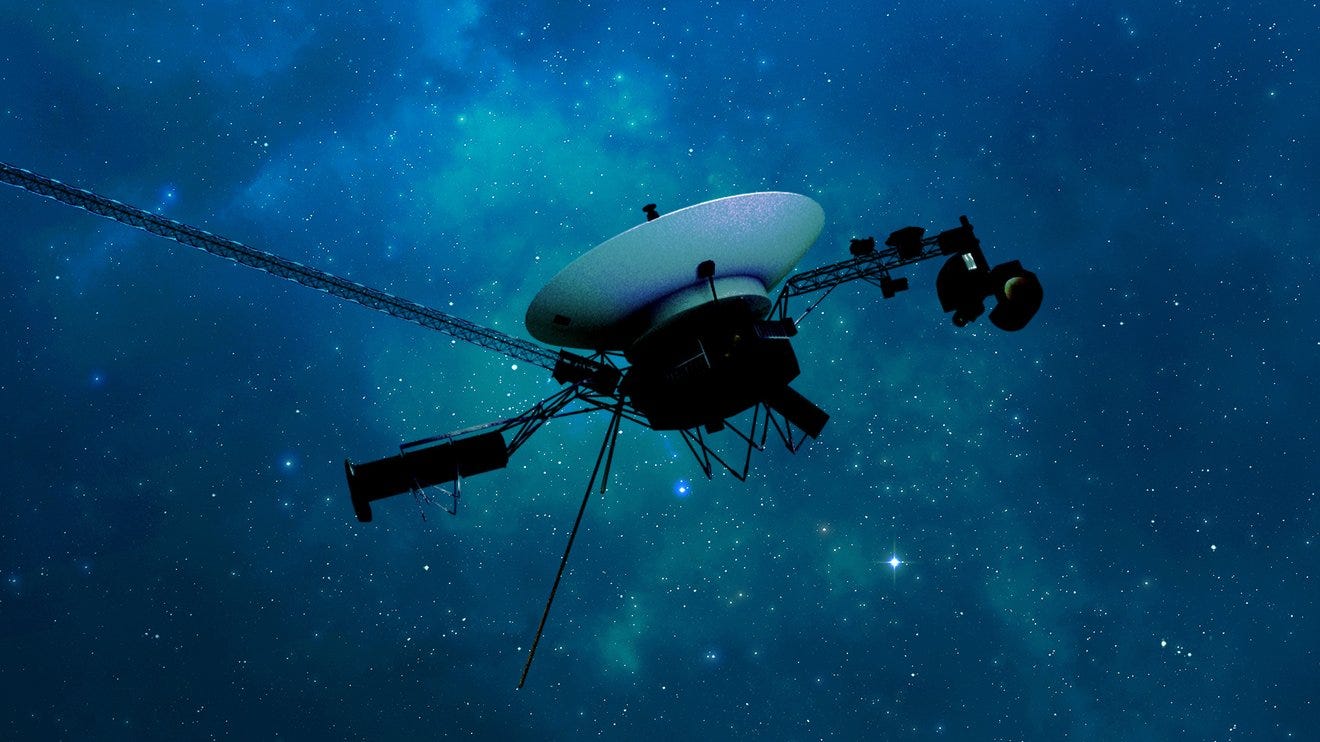
Nuclear energy has a long history in space exploration that includes applications for both power and propulsion. High energy density and reliability make nuclear an ideal candidate for supporting missions in Low Earth Orbit (LEO), cislunar space, and deep space.
The Voyager 1 and 2 spacecraft, launched in 1977, are powered by radioisotope power systems (RPSs) which convert the heat released by the decay of plutonium-238 into electricity. These nuclear generators have been operating for over four decades, enabling the Voyagers to continue sending data from interstellar space. The Curiosity and Perseverance rovers on Mars are powered by RPSs which are ideal for missions in environments with limited sunlight, such as the Martian surface.
In 1965, the first and, so far only, nuclear reactor, the SNAP-10A, was launched to space. It operated successfully for 43 days, providing 500 W of electrical power, before being shut down due to a non-reactor-related component failure.
NASA’s Kilopower project aims to develop small fission reactors capable of providing 1 to 10 KW of continuous power. Kilopower reactors could power lunar and Martian habitats, enabling long-duration missions without reliance on solar power. The Kilopower experiment successfully demonstrated a prototype in 2018, proving the concept’s feasibility.
NASA’s Lunar Surface Power Initiative, part of the Artemis program, is investing in surface nuclear power systems to provide continuous, reliable energy on the Moon. This includes collaboration with private companies to develop fission surface power systems that can be deployed as early as the late 2020s.
Developed in the 1960s and 1970s, Nuclear Engine for Rocket Vehicle Application (NERVA) was a nuclear thermal rocket engine intended to enable deep space travel (Figure 4). Several prototype engines were built and tested and included flight-ready components. By 1968, the latest iteration was deemed ready for a mission to Mars. The project was ultimately canceled in 1973, but it demonstrated the potential for nuclear thermal propulsion (NTP) to significantly reduce travel times to Mars and beyond.
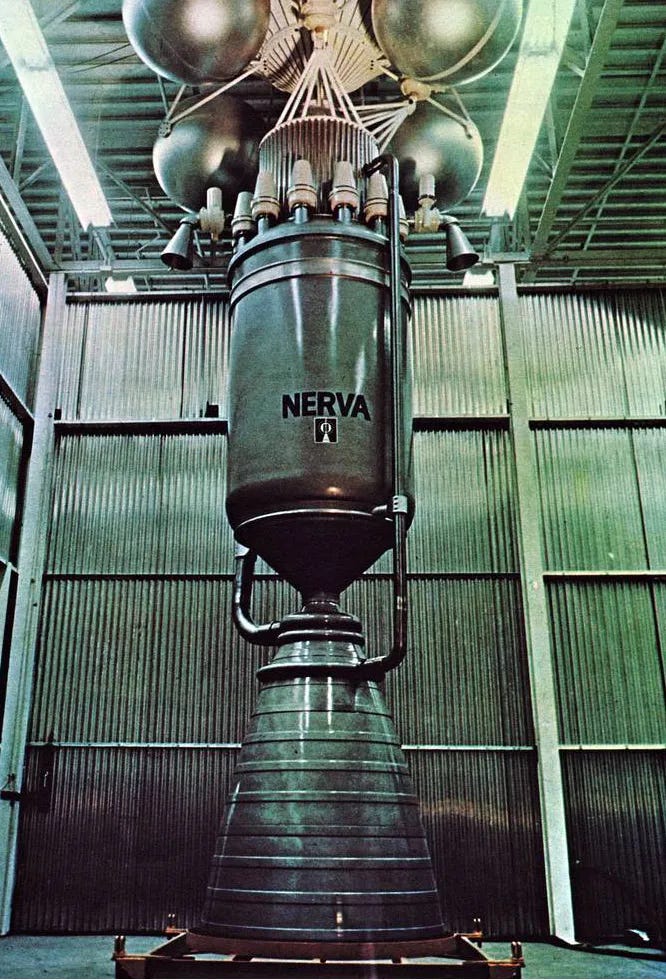
NASA’s Prometheus Project in the 2000s aimed to develop nuclear electric propulsion (NEP) systems and space reactors for deep space exploration. Although it was eventually discontinued, the research contributed valuable insights into future nuclear power and propulsion concepts.
Demonstration Rocket for Agile Cislunar Operations (DRACO), a collaboration between DARPA and NASA, is focused on reviving and developing NTP systems that could drastically reduce transit times in Cislunar space and support operations in LEO and beyond.
As missions to Mars become more ambitious, nuclear reactors and propulsion systems will play a critical role. Nuclear thermal propulsion could cut travel times to Mars by half, reducing crew exposure to space radiation. Surface fission reactors will be necessary to power habitats, life support systems, and resource utilization technologies. Nuclear energy can provide robust power and propulsion capabilities for the burgeoning cislunar economy, supporting activities such as space mining, in-space manufacturing, and satellite servicing. The reliability of nuclear power systems makes them ideal for space tugs and orbital transfer vehicles, which require continuous power and high delta-V spacecraft flight capabilities.
NUCLEAR POWER TYPES
Nuclear energy comes in three primary forms: radioisotope power systems (RPS), fission, and fusion.
• Radioisotope power systems (RPS) rely on the weak nuclearforce, which governs the radioactive decay of unstable isotopes, releasing heat that can be converted into electricity. RPS is an ideal smaller power supply for providing power in remote or harsh environments, including a proven record in deep space missions.
• Nuclear fission involves splitting heavy atomic nuclei like uranium or plutonium which releases energy through the strong nuclear force that binds protons and neutrons within the nucleus. Fission reactors are widely used in power plants on Earth and are being developed for nuclear thermal propulsion (NTP) systems to enable faster travel in space.
• Nuclear fusion merges light atomic nuclei like hydrogen isotopes using the strong nuclear force, similar to the reactions powering the Sun. If net energy gain is achieved, fusion has the potential to provide a nearly limitless source of clean energy for both power generation and advanced propulsion systems that could revolutionize space exploration.
Radioisotope Power Systems
RPS produce power by converting heat from the natural radioactive decay of isotopes into electrical energy. This process relies on the weak nuclear force, one of the four fundamental forces in nature, which governs radioactive decay. Pu-238 had typically been used due to its favorable half-life of about 87.7 years and high specific power density. Pu-238 decays primarily by emitting alpha particles (helium nuclei), which can be effectively shielded with minimal material reducing radiation hazards. The decay produces heat energy when the alpha particles slow and transfer kinetic energy to other particles they’ve collided with. The thermal power output of RPS is proportional to the decay rate of the isotope. The heat is converted to energy via a thermocouple. A thermocouple exploits the Seebeck effect, where a temperature difference across two dissimilar materials generates an electric current. These thermoelectric generators, therefore, have no moving parts, making them highly reliable and capable of long-duration operation.
Efficient heat transfer is crucial for maintaining optimal power output and preventing thermal degradation of components. RPS systems use heat pipes and thermal insulation to manage temperature gradients across the device. In the vacuum of space, radiative cooling is the primary mechanism for heat dissipation, so radiator surfaces are carefully designed. Thermoelectric generators typically achieve efficiencies of around 6-8%. Efficiency affects the overall size and weight of the RPS, which is a key constraint for space missions. More efficient, but more complex, Stirling engines have been developed and tested on earth and achieve closer to 20%.
Historic RPS applications include Voyager 1 and 2 which were launched in 1977 and have remained in operation sending data from interstellar space for over 45 years. The Curiosity and Perseverance rovers use RPS to power exploration of the surface of Mars, providing continuous power through dust storms and long Martian nights. The Apollo Lunar Surface Experiments Packages (ALSEP) used RPS units to power scientific instruments on the Moon during the Apollo missions.
RPS are ideal for powering remote sensing equipment in harsh or inaccessible environments, such as underwater autonomous vehicles or Arctic research stations, where solar and wind power are impractical.
Advances in materials science and radioisotope production are enabling the development of next-generation RPS with higher power densities and longer operational lifetimes. This could expand their use in hybrid energy systems for space habitats and combined power and propulsion systems for deep-space exploration.
Case Study: Zeno Power
Zeno Power is a US-based start-up that is reinvigorating the use of RPS by leveraging older isotope technologies and introducing novel applications using strontium-90 (Sr-90). Through innovative sourcing strategies and strategic partnerships, Zeno Power has secured key contracts with both NASA and the Department of Defense (DoD), positioning itself as a leader in the new wave of compact, reliable, and long-duration power systems.
Zeno Power was founded by a team of Vanderbilt University alumni and faculty, each bringing unique expertise to the development of innovative radioisotope power systems:
Tyler Bernstein (CEO) leads Zeno Power's strategic vision and operations. He graduated from Vanderbilt University with a B.S. in Engineering.
Jonathan Segal (COO) oversees company operations. He graduated from Vanderbilt University with a B.S. in Human and Organizational Development.
Jake Matthews (CTO) runs technical development. He graduated from the United States Military Academy at West Point with a B.S. in Mechanical Engineering and then, following service in the US Army, graduated from Vanderbilt University with a M.S. in Mechanical Engineering.
Steven Krahn (Chief Safety Adviser) serves as a Professor of the Practice of Nuclear Environmental Engineering at Vanderbilt University.
The constrained supply, high cost, and complexity of handling Pu-238 have driven interest in alternative isotopes like Sr-90, which has a more abundant supply and less stringent handling requirements. Recognizing this opportunity, Zeno Power entered the market with a mission to develop next-generation RPS based on Sr-90, a byproduct of nuclear fission that offers significant advantages in terms of availability and cost-effectiveness.
Sr-90 has a higher power density compared to other radioisotopes commonly used in RPS, making it an attractive alternative for certain applications. Zeno has developed proprietary processes to extract, purify, and encapsulate Sr-90 in a form that maximizes energy output while ensuring safety and containment, without excessive shielding requirements.
By building on legacy RPS designs that previously used Sr-90, such as early Soviet-era radioisotope generators, Zeno has minimized the need for new reactor designs, reducing time and development costs. These systems, known for their simplicity and robustness, have been updated with modern materials and control systems to improve efficiency and lifespan.
Zeno’s RPS units are designed to be modular and scalable, allowing for use in a wide range of applications, from small-scale power units for unmanned sensors to larger systems for lunar surface operations or deep-space missions.
Zeno has established partnerships with national laboratories and nuclear fuel reprocessing facilities to ensure a steady supply of Sr-90. The company has obtained the necessary licenses and regulatory approvals to handle Sr-90.
Zeno Power has successfully secured contracts including $7.5M from the DoD to demonstrate resilient distributed power on the seabed and a $30M STRATFI award to produce a flight-ready RPS-powered satellite.
Fission Power
Nuclear fission releases a large amount of energy when the nucleus of a heavy atom, such as uranium-235 (U-235), is split into smaller nuclei. Nuclear fission is one of the most efficient forms of energy production, as a small amount of fuel can release an enormous amount of energy compared to traditional fossil fuels.
Uranium is the most common fuel used in nuclear reactors, typically U-235. Natural uranium contains only about 0.7% U-235, and most reactors use Low-Enriched Uranium (LEU), where the concentration of U-235 is increased to around 3-5%. High-Assay Low-Enriched Uranium (HALEU) has a higher U-235 concentration of up to 20%. HALEU is desirable for advanced fission reactors because it is more efficient and requires fewer refueling cycles. The higher density translates to lighter weight designs which is essential for portable and space applications. The 20% limit for HALEU is intended to reduce concerns surrounding weapons proliferation (which requires 90% enrichment) but is more tightly regulated than LEU because of its higher enrichment.
Tri-structural Isotropic particle fuel (TRISO) is a new formation of (usually) uranium fuel. Each particle of fuel is coated with layers of protective materials such as carbon and ceramic-based silicon carbide which act as a containment system that prevent the release of radioactive fission products even under extreme temperatures. TRISO is extremely safe and has low potential for weapons use. TRISO is attractive for advanced nuclear designs but, at this time, is expensive.
Thorium-232 is another fertile material that can be used in nuclear reactors. Although thorium itself is not fissile, it can absorb a neutron to become Uranium-233 (U-233), a fissile isotope. Thorium is abundant and presents an alternative to uranium, especially in countries with limited uranium resources. Thorium-based reactors have been studied extensively and are considered safer in terms of proliferation risks and waste.
Reactors use coolants to transfer heat from the nuclear reactor core to a secondary system where it is used to generate electricity. The choice of coolant is crucial for reactor safety and efficiency. There are three major options: pressurized water, molten salt, and gas.
Pressurized Water Reactors (PWRs) use ordinary water under high pressure as both a coolant and a neutron moderator. The high pressure prevents water from boiling within the reactor, allowing it to absorb heat efficiently. PWRs are presently the most common type of reactor.
Molten Salt Reactors (MSRs) use a liquid mixture of salts as the coolant which can operate at higher temperatures than water-cooled reactors, improving thermal efficiency. MSRs are considered inherently safer due to their passive cooling characteristics.
Gas-Cooled Reactors (GCRs) use gases such as carbon dioxide or helium as the coolant. High-Temperature Gas-Cooled Reactors (HTGRs), a more advanced design, use helium as a coolant and TRISO fuel. HTGRs are inherently safe and efficient.
Case Study: Space Nuclear Power Corporation
The Space Nuclear Power Corporation was founded in 2020 as a spin-off from Los Alamos National Laboratory (LANL) to commercialize the aforementioned NASA Kilopower project. Kilopower was a small and lightweight fission reactor designed for power production in space and on celestial bodies like the moon and Mars. The system is designed to last for decades in extreme environments without any need for a human crew to manage it. The space reactor had been developed by the company founders while at LANL in partnership with NASA and the National Nuclear Security Administration (NNSA). The reactor produces 10 KW which was selected by NASA to power cislunar orbital craft, deep space missions, and terrestrial power production on the moon and Mars. The reactor has been tested in rigorous conditions and is ready for prototype development (TRL-5). The reactor is lightweight (around 1500 kg) and robust for launch; it is built to exceed launch safety requirements.
The company is led by industry leaders with deep expertise, and they have 18 employees.
Andy Phelps (CEO) has over 40 years of experience successfully executing large capital engineering projects across the world in the mining, construction, and nuclear industries. He completed a White House fellowship with the Department of Defense and was an Associate Director at LANL. He has a BS in Civil Engineering from the Georgia Institute of Technology.
Patrick McClure (COO) was the project lead for Kilopower at LANL. He is a pioneer in very small reactor design and an expert in nuclear safety analysis and regulatory management. He has a BS in Petroleum Engineering from the University of Oklahoma and a MS in Mechanical Engineering from the University of New Mexico.
David Poston (CTO) was chief reactor designer for Kilopower at LANL including for the successful DUFF and KRUSTY reactor tests. He has also developed several nuclear thermal propulsion (NTP) design concepts for NASA. He has developed several special purpose reactor concepts for commercial and defense applications, ranging from 1 kW to 10 MW power. He has a BS in Mechanical Engineering from the University of Michigan, a MS in Mechanical Engineering from Stanford University, a MS in Nuclear Engineering from the University of California at Berkeley, and a PhD in Nuclear Engineering (with a thesis on NTP) from the University of Michigan.
Marc Gibson (Chief Engineer) was the lead engineer for the Kilopower project at NASA. nuclear systems Kilopower project tasked with advancing the technology readiness of fission power systems for space. At NASA, he was responsible for the engineering and development of nuclear systems for in-space and planetary surface power. Prior to NASA, he had been chief engineer for numerous commercial and government research projects. He has a BS in Mechanical Engineering from the University of Akron and a MS in Aerospace Engineering from the Case Western Reserve University.
They are executing a $33.7M contract for the USSF Joint Emergent Technology Supplying On-orbit Nuclear Power (JETSON) in partnership with Lockheed Martin Corporation and BWXT. The JETSON project is to build a reactor to support a craft in rectilinear orbits about the moon, powering all equipment to surveil the entire moon surface including avionics and ion thrusters.
The JETSON system utilizes a compact fission reactor that generates heat, which is then converted into electrical power using Stirling engines. This design is based on the successful Kilopower Reactor Using Stirling Technology (KRUSTY) demonstration conducted by NASA and the Department of Energy in 2018. The reactor is designed to produce between 6 kilowatts-electric (kWe) and 20 kWe of power, significantly surpassing the capabilities of conventional solar arrays and enabling sustained operations in shadowed or deep-space environments where solar energy is insufficient.
Fusion Power
Fusion, the process that powers the stars, is when light atomic nuclei hydrogen isotopes (often deuterium and tritium) fuse to form heavier nuclei and release large amounts of energy in the process.
The main challenge in harnessing fusion for power lies in achieving and maintaining the extremely high temperatures and pressures required for the fusion of nuclei. This process occurs in the plasma state, where the fuel is heated to millions of degrees. Magnetic confinement (used in tokamaks like ITER) and inertial confinement (used in laser-based fusion like at the National Ignition Facility) are the two primary approaches being developed.
In magnetic confinement, powerful magnetic fields hold the hot plasma in place to prevent it from touching reactor walls, while in inertial confinement, lasers compress the fuel to extreme densities and temperatures, igniting fusion.
While controlled fusion has not yet achieved sustained net-positive energy production, recent advances, such as the experimental progress at ITER and private companies with significant capital investment working on smaller designs bring hope that fusion could become a viable source of clean and nearly limitless energy in the coming decades.
Although fusion for power production is still under development, fusion technology already has practical applications. One such application is the production of neutron sources. Fusion reactions produce high-energy neutrons, which are useful for non-destructive testing (NDT) of materials, such as inspecting aircraft parts, bridges, or other critical infrastructure. Fusion neutron sources are also important in medical applications, particularly for generating neutrons in experimental cancer treatments like boron neutron capture therapy (BNCT).
Case Study: Avalanche Energy
Avalanche Energy was founded in 2018 to create compact, scalable fusion reactors for remote or distributed locations.
The company was founded by two former Blue Origin engineers:
Robin Langtry (CEO) developed advanced technologies at Blue Origin and Boeing. He graduated from the University of Stuttgart with a PhD in Mechanical Engineering from
Brian Riordan (COO) has extensive experience in mechanical design and IP development. He was previously as a principal engineer with Blue Origin.
Avalanche’s approach involves the rapid iteration engineering approach applied to micro-fusion reactors. The small size allows iterative prototyping which can provide a significant advantage in development timelines. The system is designed to run on deuterium, a stable isotope of hydrogen, offering a cleaner and safer fusion reaction compared to traditional nuclear energy. The reactors are compact enough to be used in space exploration, military operations, and remote industrial sites.
Avalanche Energy’s micro-fusion reactor is called the Orbitron and employs electrostatic fields to confine fusion ions in a small vacuum chamber while using a magnetron to achieve high ion densities. This configuration allows them to achieve fusion at relatively low voltages compared to other approaches, like large tokamaks, which use massive magnets or lasers. The primary fusion fuel in the reactor is deuterium and tritium, and the resulting reaction releases neutrons that generate heat, which can be converted into electricity. The aim is to produce 1 to 100 KW in a portable reactor.
Avalanche Energy’s target market includes defense applications where portable energy sources are essential for forward operations. An example would be replacing a diesel generator and eliminating the need for fuel resupply convoys. The new space industry could use Avalanche’s reactors to power long-duration missions or extraterrestrial bases. The company’s technology could also play a vital role in energy-intensive industries, such as mining and telecommunications, where reliable off -grid power is needed.
Avalanche Energy has raised about $45 million and has also secured contracts with the U.S. Department of Defense to explore the military applications of its fusion technology including a Defense Innovation Unit (DIU) award for nuclear-powered spacecraft design.
Fission for Space Propulsion
Nuclear fission has been prototyped as a method for space propulsion and has recently been taken up again by NASA. Nuclear can provide large amounts of energy over long durations, far exceeding the capabilities of conventional chemical propulsion. Two primary concepts using fission for space propulsion are Nuclear Thermal Propulsion (NTP) and Nuclear Electric Propulsion (NEP).
In NTP, a fission reactor heats a propellant, typically hydrogen, to extremely high temperatures. The heated propellant expands rapidly and is expelled through a nozzle to generate thrust. The reactor is typical fueled by U-235 and has liquid hydrogen passed through the core where it is heated to temperatures in excess of 2,700 K. The hydrogen expands and is expelled at high velocity through a rocket nozzle. NTP systems offer a specific impulse of around 800-900 seconds, which is more than double that of conventional chemical rockets. This high efficiency allows for faster travel times and reduced propellant requirements. The high efficiency and thrust provided by NTP systems can reduce travel time to Mars from about 9 months (using chemical propulsion) to as little as 3-4 months, minimizing crew exposure to space radiation and reducing mission risk. NTP is ideal for long-duration missions, as it provides a sustained power source without the need for large quantities of chemical propellant/refueling.
NEP systems use a nuclear reactor to generate electrical power, which is then used to power electric thrusters such as ion engines or Hall-eff ect thrusters. In this case, the thermal energy of the reactor is used to produce electricity through a thermionic converter or Brayton cycle generator. The electricity powers an electric propulsion system that ionizes a propellant (usually xenon gas) and expels the ionized particles at high speeds creating thrust. Although NEP is efficient, the thrust it produces is relatively small, which limits its usefulness for rapid maneuvers or missions requiring high initial acceleration. NEP is ideal for satellites and deep space missions where continuous low-thrust propulsion can be applied over long periods.
Case Study: Dark Fission
Dark Fission Space Systems was established in 2022 to pioneer the development of commercial nuclear thermal propulsion (NTP) rocket engines aimed at revolutionizing space propulsion for cislunar missions and beyond.
The founding team comes with deep engineering, space, and nuclear experience.
Fred Kennedy (CEO) previously served as the President of Momentus, a company specializing in space tug technology. He was Vice President for Future Missions at Astra, focusing on small satellite production capabilities. He founded the US DOD Space Development Agency (SDA). He served for 23 years in the US Air Force with roles at the White House, Pentagon, and National Reconnaissance Office. He graduated from the University of Surrey with a PhD in Electronics and Physical Sciences.
Michael Jacox (President) was Vice President of Engineering at Space Micro and had previously founded StarVision Technologies. He was Deputy Director of the Spacecraft Technology Center at Texas A&M University. He led the Air Force Research Laboratory’s Solar Bimodal demonstration program and managed space nuclear systems development at the Idaho National Laboratory. Graduated from the Georgia Institute of Technology with a BS, The University of Idaho with a MS in Nuclear Engineering, and Texas A&M University with an MBA.
Greg Loboda (COO) was President and Owner of Sirius Partners LLC. He had held critical engineering and manufacturing leadership roles for multiple aerospace startups, including Universal Hydrogen and Momentus. He was Vice President of Engineering at Vector Launch, directing the development of the Vector-R launch system, and held leadership positions at SARA, Kratos Unmanned Aerial Systems, and Raytheon. He graduated with BS and MS degrees in Aerospace Engineering from the Massachusetts Institute of Technology.
Dark Fission Space Systems aims to launch an orbital demonstration vehicle powered by an NTR engine within five years of its founding, intending to transform in-space propulsion by providing ultralightweight, powerful, and efficient engines. The company's technology leverages decades of development in nuclear systems and space propulsion, focusing on enabling rapid and cost-effective transit within the cislunar space and beyond.
By harnessing nuclear thermal propulsion, Dark Fission seeks to open new frontiers in space exploration and commerce, offering innovative solutions for government and commercial partners operating in geosynchronous Earth orbit (GEO) and the broader Earth-Moon system.
Fusion for Space Propulsion
Fusion’s potential for space propulsion is based on its ability to generate massive amounts of energy from a small amount of fuel. Fusion propulsion concepts include designs like the Direct Fusion Drive (DFD), which would use a fusion reaction to heat a working fluid (e.g., helium or hydrogen), expelling it through a nozzle to generate thrust. This system could theoretically provide both high specific impulse (efficiency) and significant thrust, making it ideal for long-duration space missions, such as crewed missions to Mars or even beyond.
Case Study: Helicity Space
Helicity Space was founded in 2018 by Marta Calvo, Dr. Setthivoine You, and Dr. Stephane Lintner. Dr. Lintner has a doctorate in applied mathematics from Caltech and was a managing director at Goldman Sachs. Dr. You is a plasma physicist from with extensive research experience in fusion technologies. Ms. Calvo is a US Army veteran with experience in aerospace quality assurance.
Helicity Space secured $5 million in seed funding in December 2023 and later, undisclosed investment in April 2024.
The company is developing the Helicity Drive, a fusion-based propulsion engine designed for deep space missions. This novel propulsion system leverages plasma jets, magnetic reconnection, and peristaltic magnetic compression to achieve fusion reactions, aiming to make space travel faster, safer, and more fuel-efficient. Helicity Space is closely aligned with Lockheed Martin through both funding and technical collaboration. This partnership provides access to Lockheed’s research capabilities, potentially accelerating Helicity’s development efforts.
Helicity’s long-term goal is to advance fusion propulsion technology to enable sustained deep space exploration, aligning with broader ambitions to support future space economies and operations beyond Earth’s orbit and into cislunar space.